Let me take you back 2400 years to ancient Greece. The philosopher Plato is thinking about the universe. Fundamentally it has to be built of simple objects, he muses. The simplest 3D objects are the most symmetrical ones. So he associates each basic element (fire, water, air, earth and aether) with a regular solid.

Of course, we don’t believe in Plato’s theory today. Nevertheless it’s a fascinating example of the interplay between maths and physics. This dialogue has continued down the ages. For more than two millenia we’ve been striving to arrive at a mathematical understanding of reality.
But what’s the point? On the one hand, there’s the philosophical argument of Plato. Somehow mathematics seems like the fundamental language of the universe. We’d better speak it if we’re to hold a meaningful conversation about physics. But there’s a more prosaic reason too.
Maths is simply the most efficient way of encoding our predictions about experiments. Centuries of science have shown that expressing models mathematically enables swifter and more precise verification of our ideas. It seems then that maths and physics are inextricably linked.
For PhD students and layman alike, this realization is a terrifying one! Pretty much everyone finds mathematics hard. Symbols and equations are easy to muddle up. Calculations are opaque at best, and impossible at worst. Even the basic formula for the current understanding of particle physics is a daunting thicket of letters.

But now I’m going to make a bold assertion. Actually the maths is quite easy. It’s the physics that’s hard. Really maths is just a collection of interesting abstract ideas. Most of these are motivated by some natural intuition. This makes them easy to “get” on a gut-instinct level. The problems arise when you have to select the right mathematical ideas and apply them to the real world.
I’m going to give you three brief examples of big mathematical ideas in modern physics. You’ll see that the concepts themselves are beautifully simple. The scary mess of symbols only rears it’s ugly head once you bolt these ideas together with physics.
1. Symmetry
Most of us have an intuitive idea about symmetry. You’d say something’s symmetric if it’s the same after you rotate or reflect it. In many cultures objects and patterns with lots of symmetry are considered beautiful. That’s principally because humans are lazy.
We like symmetrical things because they’re simple. A snowflake is easy on the eye because it’s very regular. Look at just a small part of it and you can predict the rest. So symmetry provides us with useful information for free.

You can see why mathematicians and physicists like symmetry – it makes their job easier! The more symmetry you claim to have, the more constrained your answers must be. That’s all very well as an abstract principle perhaps, but where does the physics come in?
Fortunately the real world has lots of symmetry. Look to your left. Have the laws of physics changed? Hopefully not! This tells us that physics has a rotational symmetry. When you walk to the shops reality doesn’t suddenly shift. So there’s a so-called translational symmetry too.
From our experience with the snowflake we should be able to extract some information about the world. We need to get a feel for what that should be.
Imagine that walk to the shops again. You’re pretty meticulous so you always walk at the same speed. And you like some variety so you’ve got a choice of two routes. Each route is exactly a mile long.
It’s obvious that your journey will take the same time whichever route you take. That is providing there’s nothing special to distract you on the way. Perhaps one route has a travelator, so you go faster even though you aren’t pacing quicker. Maybe on the other route you bump into an old friend and stop for a chat.
But in both of these scenarios you’re changing your overall speed. In the absence of such distractions the speed you walk is conserved, precisely because there’s nothing special about either route you take. In other words the translational symmetry gives rise to a speed conservation law.
So spatial symmetry gives rise to speed conservation. More precisely physicists talk about momentum conservation, which takes into account mass as well. What about rotations? Well they lead to conservation of angular momentum. You’ll have felt its effects if you’ve ever been on a rollercoaster!

So we’ve got a double whammy – symmetry exists in the real world, and it makes doing physics easier. It’s no wonder that our best theory of particles (the boringly-named “Standard Model”) is based entirely on symmetries.
Modern physics has all kinds of more general symmetries. Supersymmetry, gauge symmetry, colour symmetry, flavour symmetry – the list goes on! These mathematical principles guide us towards particular models of reality. In fact the terms in the daunting formula above are really just a consequence of choosing appropriate physical symmetries.
Now for a quick tour of the current battle-lines. Lately people have discovered new symmetries related to particle scattering. Others have been able to exploit these to get a better perspective on the theoretical foundations.
The upshot is a symmetrical jewel which encodes a huge amount of information. This amplituhedron is something like Plato’s solids. So maybe the Greek geezer wasn’t so wrong after all!
2. Integrability
Unlike symmetry, you’ve probably never heard of integrability. At heart it’s just a posh word for something extremely simple. We say a physical model is integrable if you can get exact answers from it.
This seems a little arcane, until you realise that we only have approximate solutions to most physical problems. Unfortunately the equations that describe reality are pretty hard. So physicists have to estimate the answer using various techniques. Only in exceptional cases can we do a full mathematical derivation.
This malarkey started off with Newton. In his famous Second Law he claimed that force was proportional to acceleration. This is immediately obvious during takeoff in an aeroplane, for example.
Many of you will know that acceleration measures how fast your speed is changing. So if you know the force being applied, Newton is telling you how the speed changes. Mathematicians call this a differential equation since difference is a synonym for change.
The usual way of solving these equations is called integration. Suppose you know the speed at the start of the motion. Newton gives you the change in speed from one moment to the next. Adding up all the changes over these tiny moments gives you the speed at any point in the future!
Sadly, integration gets really tricky for complicated systems. That’s when the physicists have to approximate things. The most famous example dates back to Newton’s time. Suppose you want to understand the motion of three planets interacting gravitationally. Nobody’s ever been able to find an exact solution for this, and there’s strong evidence that one doesn’t exist at all!
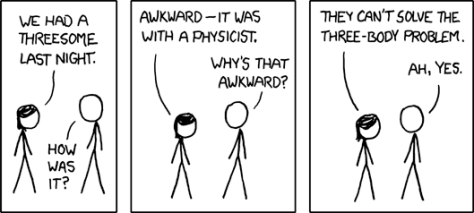
The idea of integrability carries over into quantum mechanics too. Basically a quantum theory separates reality out into a spectrum of chunks. In particular, energy comes in discrete lumps called quanta. But finding out what energies are allowed is difficult. Again you’ve got to solve a differential equation!
In general physicists make approximations, usually by perturbing a system slightly from some ideal configuration. This works remarkably well, but can’t give us complete knowledge of a theory. This ignorance is one of the difficulties associated with forming a quantum theory of gravity.
It seems that our mathematical accounts of nature are just not integrable. Neither in Newtonian physics, nor on the quantum level do the complicated interactions admit a pretty solution. In fact, integrability is a rare and magical property.
Typically integrability is associated with high symmetry situations. We saw in the last part that symmetry gives us useful constraints on allowed physics. By adding a healthy tablespoon of symmetry to the mix we raise our chances of finding a neat solution. Such souped-up theories are an active area of research.
But what’s the point? If integrable theories aren’t very realistic, then why should we be looking at them. Quite apart from their mathematical elegance, they give us new insights into the structure of our model. Methods that start off in the highfalutin realms of an integrable theory can percolate down to more realistic descriptions.
That’s just what some people hope will happen for our modern day Platonic amplituhedron. Many regard it’s beauty as a remnant of the integrability of a relevant souped-up theory. But its lessons could well inform our view of the universe.

3. Duality
Racing into the final furlong, we’re confronted with another unfamiliar word. But you might have an inkling about what it means. Think of your favourite dynamic duo – two individuals that present different perspectives yet work well together. From Bush and Blair to Ant and Dec, these relationships are common in the celebrity world.

Curiously this kind of friendship crops up mathematically too! Here’s an easy example. Suppose you draw two random lines on a piece of paper. If your paper is big enough they’ll meet at a point. Similarly if you put two dots on your paper there’s a single line that joins them.
Writing this down as a formula we can roughly say
So if you prove something about points, you can immediately translate it into a statement about lines and vice versa. This is a simple example of a mathematical duality. You’ve got two different perspectives on geometry that work together to enrich your understanding.
You’d be forgiven for finding that example unremarkable. There’s nothing very exciting about lines on bits of paper. So let me convince you that duality is important in the real world.
Suppose you’re sitting at your computer, listening to your favourite song. Chances are that it’s been compressed into MP3 format. Have you ever wondered how that’s done? You’ve got to take the wave encoding the song and smush it together somehow.
It’s hard to work out a procedure at first. Just putting all the wave peaks closer together won’t work because that’ll just raise the pitch of the whole song! The key is to come at the issue from a different perspective. You decompose the song into different frequencies and then remove pitches that a human can’t hear.
The trick we used was to exploit a duality between time and frequency. Mathematically the act of decomposing the song is called a Fourier transform. This provides a new perspective on the original wave. You can transform things back and forth as you wish depending on which viewpoint is most convenient.
Duality is convenient because it gives us several mathematical models reproducing the same physics. You’re free to analyse the theory from whatever perspective is the most appealing. We’ve seen that our models are generally very hard to solve. Duality can help to simplify the situation.
Physicists exploit duality in two main ways. Many dualities allow you to improve your approximations of a system. Others give you further details of hidden solutions or symmetries. Those which do both are especially highly prized.
We’ll finish with a modern duality which remains a hot topic today. It has been instrumental in improving our knowledge of several physical models. More specifically it relates a souped-up quantum theory to a stringy theory of gravity. We call it the AdS/CFT duality.
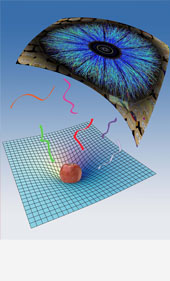
This duality is a mathematical chocolate ganache – decadent, moreish and extremely rich. Many of these qualities arise through large amounts of symmetry. Each of the AdS and CFT duo are exquisitely symmetrical and perhaps even integrable.
The heady mixture of duality, symmetry and integrability has inspired much research over the past 15 years. In many ways, the amplituhedron is the grandchild of this cross-fertilisation. And the story is similar elsewhere in physics. From cosmologists to materials scientists, everyone’s using this powerful brew.
So get out there and impress your friends! I’ve given you a toolbox of ideas from the forefront of theoretical research. In a sense, you are right at the cutting edge. Though you might need a bigger instruction set before you try to build anything.